Atomic Clocks
Atomic clocks allow us to precisely define and measure time, and they are crucial aspects in the functioning of our daily lives.
Reading Time: 4 minutes
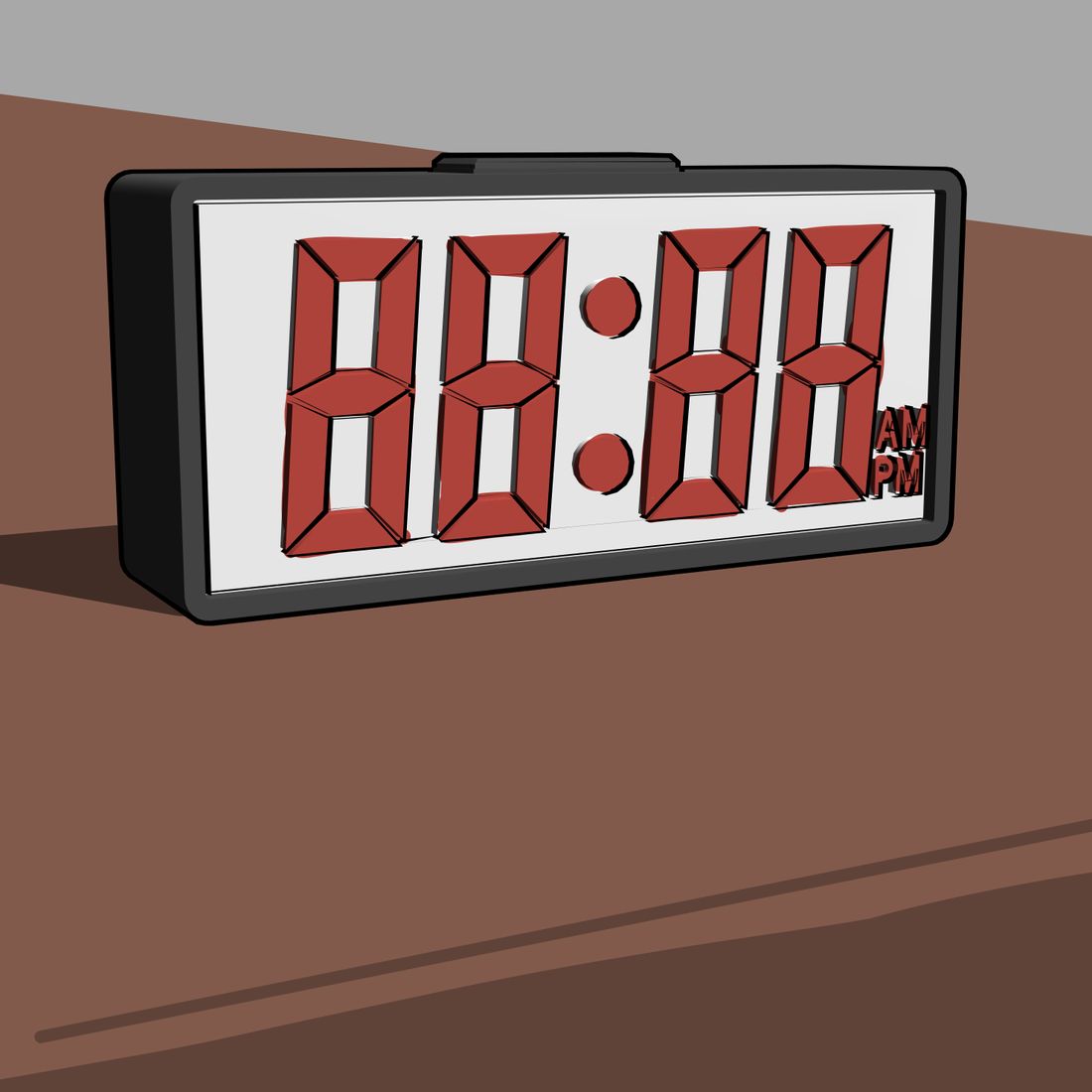
Whether scheduling an appointment, running to class at 7:58 a.m., or catching a flight, our lives run on the clock. We rely heavily on timekeeping to ensure that we all get things done at the same—universal time. But how does everyone agree on a common time? Traditionally, time has been a very local concept, driven by the position of the sun in the sky. However, as globalization has necessitated time synchronization across long distances, we’ve had to adopt a more globally accepted measure of time.
In 1656, Dutch mathematician and astronomer Christiaan Huygens invented the first mechanical clock that relied on the predictable oscillations of a pendulum. His invention was highly influential, reducing timekeeping error to about 15 seconds a year—around 60 times more accurate than the prevailing technology at the time, which relied on wide pendulum swings of up to 100 degrees. Still, modern timekeeping demands a much higher level of precision to coordinate communication across a wide variety of devices and networks. For example, Global Positioning Service devices rely on accurately measuring the amount of time it takes a signal to travel from a satellite to Earth. Being off by even a millisecond can lead to errors of up to 300 kilometers—roughly the distance between New York City and Washington D.C.
Enter the atomic clock, which allows us to measure time with an error rate of only one second per 158 million years. The phase transitions of cesium atoms have several characteristics that make them effective as atomic clocks.
A cesium atom has a single valence electron in its outermost shell, which is the only unpaired electron in the entire atom. The nucleus of an atom and each electron can spin in two possible directions, and the unpaired electron of cesium can have one of two spin states—one in which the nucleus and valence electrons spin in the same direction or one in which the two spin in opposite directions. When both the nucleus and electron spin in the same direction, the cesium atom is high-energy, and when they spin in opposite directions, the atom is low-energy. When a cesium atom in the low-energy state is exposed to electromagnetic radiation—waves in an electromagnetic field—of a specific frequency, it can switch from the low-energy to high-energy state.
In an atomic clock, a stream of vaporized cesium atoms pass through a magnetic field, separating the two cesium spin states. Since atoms in low-energy and high-energy states have different spins, the magnetic field deflects them in different directions. This allows the clock to separate the low and high-energy atoms into two different streams. Next, a device called an oscillator subjects the stream of low-energy atoms to electromagnetic waves at the exact frequency that causes them to switch to the high-energy state. The resultant stream is again filtered through a similar magnetic field. Finally, a detector finds the proportion of atoms that switched state and adjusts the oscillator’s wavelength to maximize the production of high-energy atoms. This process keeps the oscillator fine-tuned to the exact frequency that causes the state switch in cesium atoms.
This process results in the oscillator producing electromagnetic waves at a consistent frequency: 9,192,631,770 oscillations, or cycles, per second. An atomic clock measures the total number of oscillations by counting the consecutive peaks or troughs of the electromagnetic waves the oscillator generates.
More recent cesium clocks—fountain clocks—are designed to be even more precise. For example, the NIST-F2 clock can have an error rate as low as one second per 300 million years. Fountain clocks have a slightly different mechanism that focuses on reducing thermal noise—measurement error induced by atom movement—to improve measurement accuracy. Lasers cool cesium atoms in a vertical chamber to temperatures close to absolute zero—the lowest theoretical possible temperature—reducing kinetic energy and thermal noise. Another set of lasers throws the atoms up the vertical chamber through a microwave oscillator. As the atoms fall due to gravity, they go through the oscillator again, where some of the low-energy state atoms switch to high-energy states. As the high-energy atoms switch back to low-energy, they emit light, which is detected by a sensor in order to fine tune the oscillator.
Although cesium clocks already provide a very accurate measurement of time, scientists are working on developing even more specific clocks. Strontium-based atomic clocks—which function in the same way as cesium-based atomic clocks but rely on energy transitions in strontium atoms—are expected to have an error of at most one second every 15 billion years.
Clocks that provide us with such levels of accuracy are promising developments for space communication. Currently, satellites and spacecrafts rely on atomic clocks to precisely coordinate with control centers on Earth. Precise measurement of time makes it possible for experts to correctly locate and send appropriate instructions to spacecraft, allowing the spacecraft to navigate with extreme precision. As even more accurate clocks—such as the strontium-based atomic clock—are developed, we will likely be able to communicate and synchronize space and time coordinates with devices that are light years away.